On September 14 2015 the first direct observation of a gravitational wave was made at the LIGO Hanford and Livingston observatories. This event, named GW150914, was emitted by two black holes, each around 30 times the mass of the Sun, merging around a billion light years away. We have recently published a paper giving details about how GW150914 was found and confirmed in LIGO’s data.
The LIGO observatories consist of two perpendicular arms, each 4km in length, and use lasers travelling along the arms to measure tiny changes in their length. When a gravitational wave passes through a detector it stretches and squeezes space by a very small amount and it was by observing this effect that LIGO detected GW150914.
But how does LIGO go from these very precise distance measurements to confirmation of a detection?
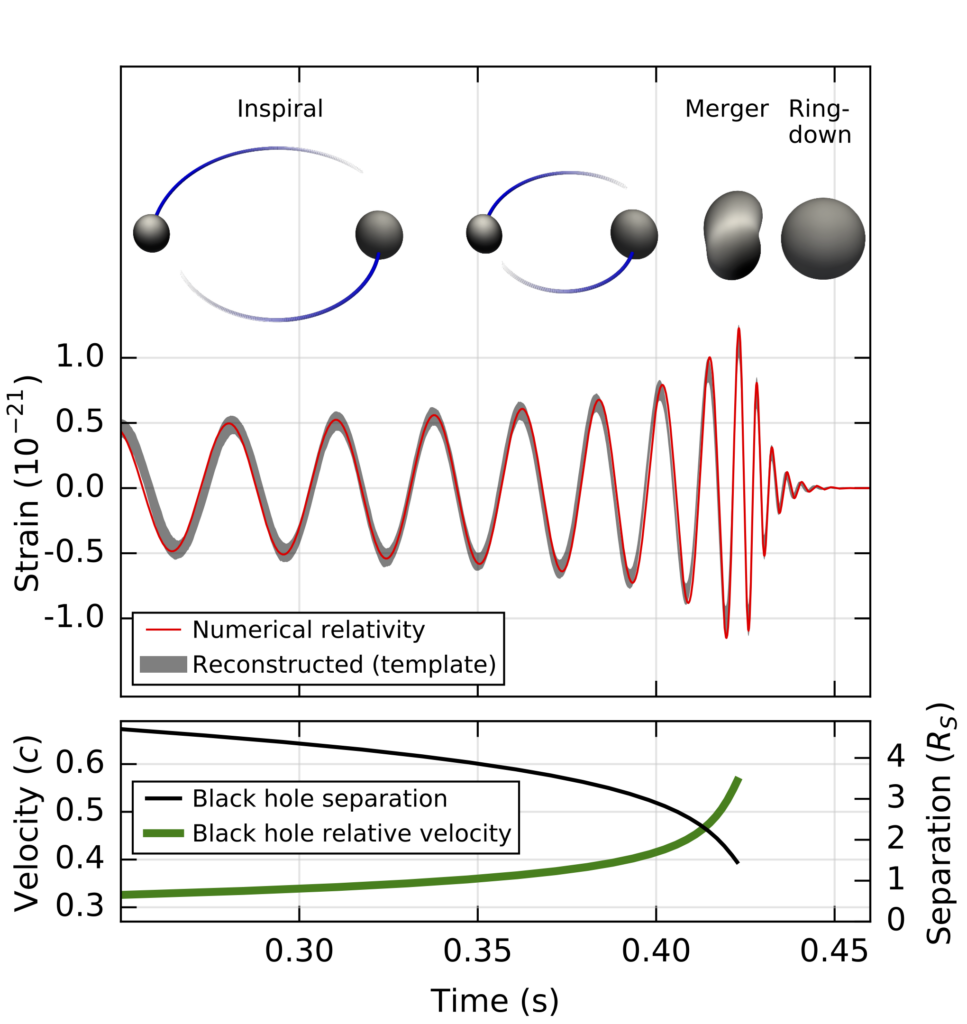
Figure 1. What a gravitational wave looks like in the time domain (middle), comparing the reconstructed gravitational-wave strain (as seen at Hanford) with the predictions of the best-matching waveform computed from general relativity. Also shown is what is happening to the black holes (top) and the separation and velocities of the black holes (bottom).
Matched Filtering
The initial detection was made by a so-called ‘burst’ search, which searches for any signal that has the general pattern of a gravitational wave. We know that a gravitational-wave signal from two compact objects (black holes or neutron stars) merging should increase in frequency and strength as the objects approach each other; this is known as a ‘chirp’ signal. Instead of looking for any chirp-like signal we can also compare our data to carefully calculated templates. The shape of a chirp signal depends on the system’s properties – the most important being the masses of the objects and how much they are spinning.
A technique known as matched filtering is used to see if there are any signals contained within our data. The aim of matched filtering is to see if the data contains any signals similar to a template bank member. Since our templates should describe the gravitational waveforms for the range of different merging systems that we expect to be able to see, any sufficiently loud signal should be found by this method. A true signal should also cause a ‘match’ in both detectors within the time it takes to travel between them. Since we know gravitational waves travel at the speed of light we can calculate this time: around 15 milliseconds or less (depending on the direction on the sky from which the gravitational-wave signal emanates).
We used two separate matched filtering pipelines, called PyCBC and GstLAL, to analyze LIGO data collected from September 12 to October 20 2015. During these 38.6 days there was around 17.5 days when the two LIGO were operating simultaneously and giving high quality data that we could analyze.
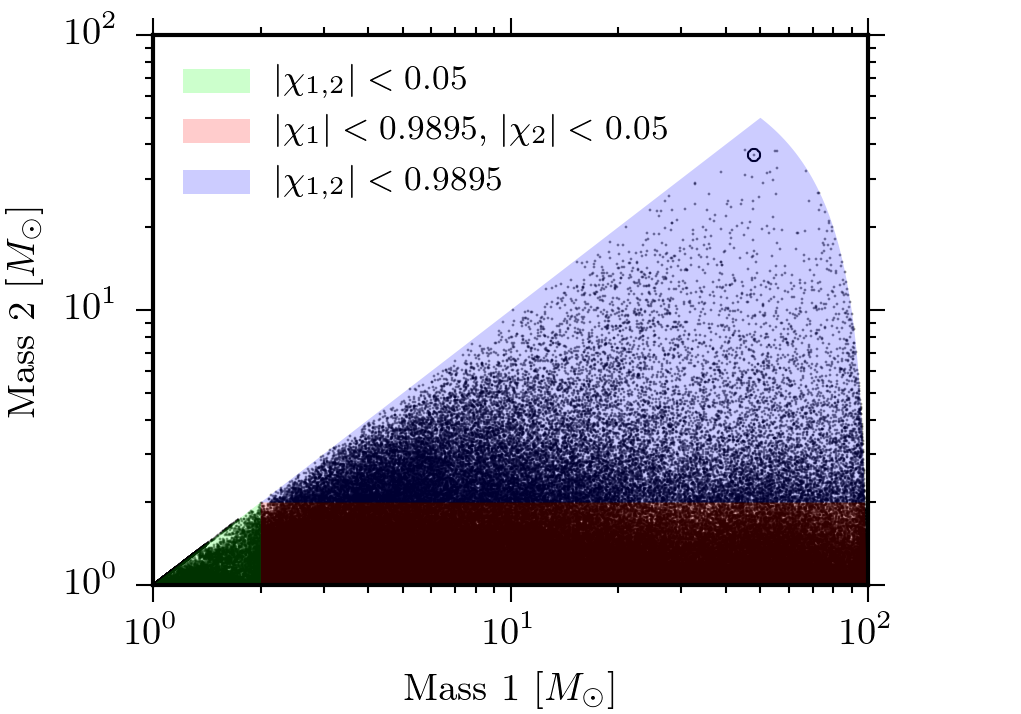
Figure 2. The template bank used in LIGO’s matched filtering searches. The circle shows the template best matched with GW150914.
PyCBC
PyCBC carries out matched filtering using gravitational waveforms as a function of frequency, and calculates a signal-to-noise ratio (SNR) for each detector and each template. The SNR is a measure of how loud an event is compared to the background noise of the detector. Any portions of data that have a high enough SNR then go through what is called a ‘chi-squared’ test. This is necessary as instrumental ‘glitches’ can often give high values of SNR but do so over a narrow range of frequencies. We check that the SNR comes from the correct range of frequencies for a real signal, and not from such a glitch.
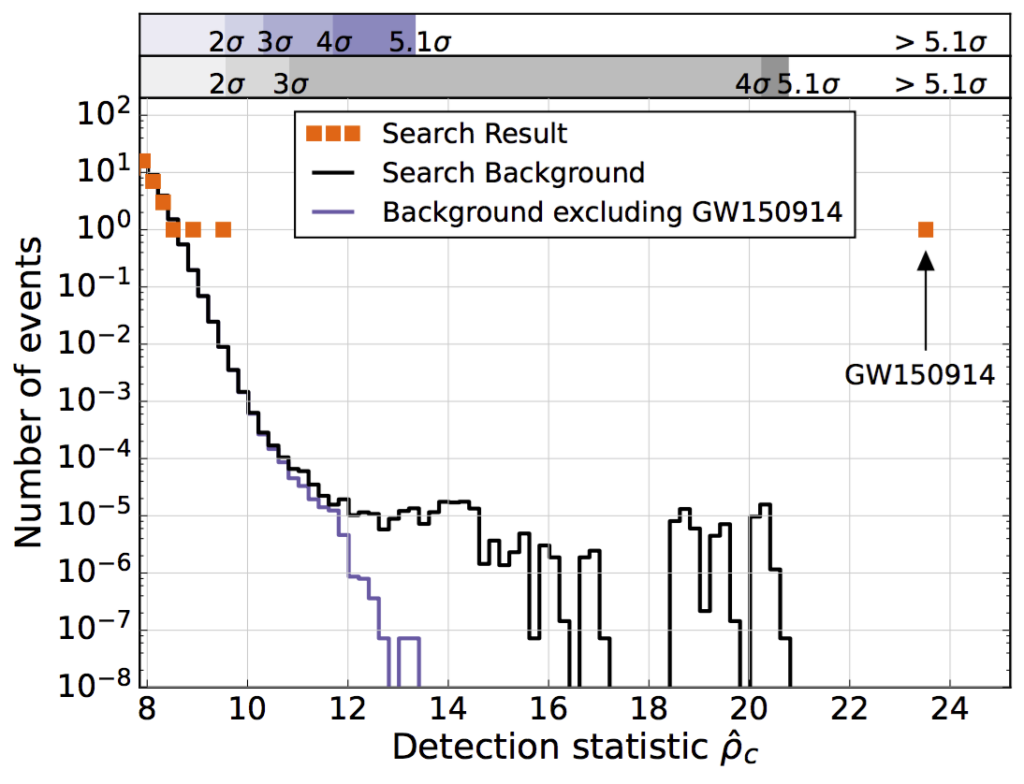
Figure 3. Search results from PyCBC analysis. The x axis is a measure of how loud an event is. The lines show how many events of each loudness we expect to see if only background noise is present. The orange squares show that we saw many relatively quiet events that match our background predictions but that GW150914 is very, very unlikely to have been caused by noise – less than one occurrence in about 200,000 years of data like this – a value that corresponds to a detection significance of more than 5 “sigma”.
GstLAL
Unlike PyCBC, the GstLAL conducts matched filtering based on the time dependence of the waveform templates, using techniques developed in order to allow searches to be carried out near real-time. Much like PyCBC, GstLAL finds any sections of data with high SNR and subsequently a quantity xi-squared is calculated: this serves the same purpose as the chi-squared test used in the PyCBC analysis.
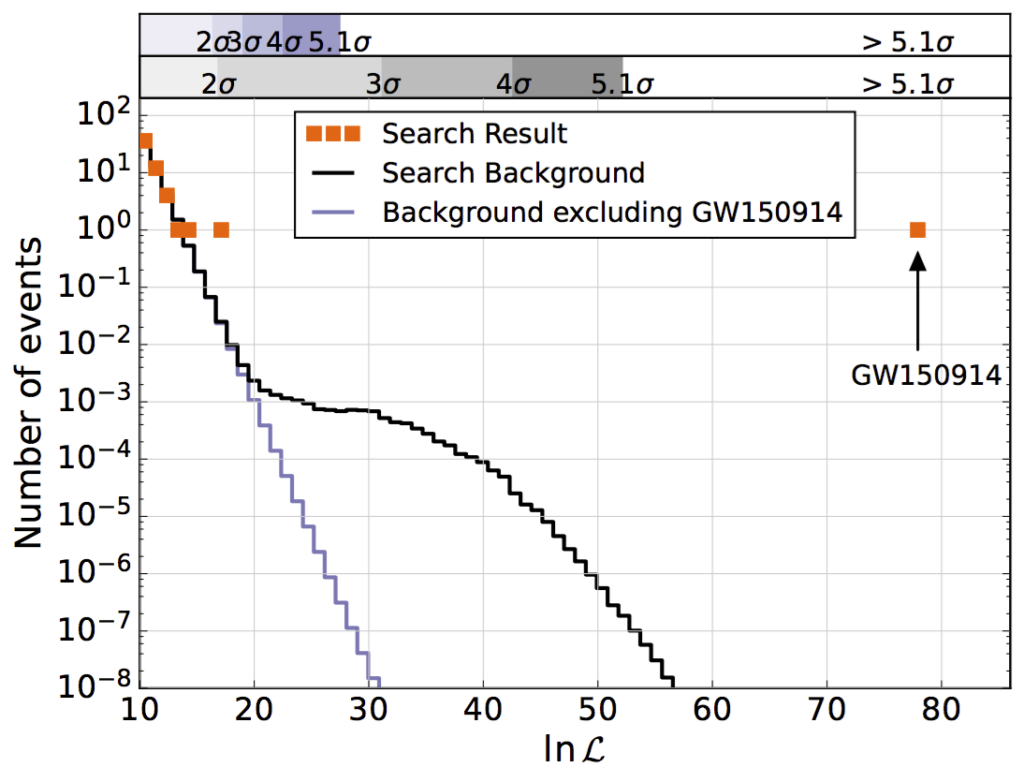
Figure 4. Search results from GstLAL analysis. This plot is very similar to the PyCBC search results except that GstLAL uses a different quantity to measure how loud an event is. It again shows that GW150914 is almost certainly a real astrophysical source.
Search Results
GW150914 was observed as the most significant event in both analyses. It was found to be best-matched by the same template in both analyses with masses of 47.9 Solar masses and 36.6 Solar masses and effective spin of 0.2. To get a better picture of what kind of system we detected, we need to perform follow-up parameter estimation on the signal. Table 1 of our paper presents the mass estimates obtained through this subsequent analysis. More information on parameter estimation can be found in this science summary.
In addition to GW150914, a second candidate event was recorded: LVT151012. This event had an SNR of 9.6 with the PyCBC analysis, and detector characterization studies have not identified any instrumental or environmental artefact as having been responsible for this event. However, we can estimate a “false alarm probability” (FAP) for the candidate, which seeks to measure the probability that a random noise fluctuation in the detector (i.e. a ‘false alarm’ ) could mimic a real gravitational-wave signal with this SNR. From the PyCBC and GstLAL analyses we estimated a FAP of about 2% and 5% respectively. In neither case was this FAP sufficiently low to confidently claim the event as a real gravitational-wave signal. If LVT151012 is of astrophysical origin its properties can be determined through parameter estimation, and these are also shown in table 1 of our paper.
We have shown, to a very high certainty, that GW150914 was a real gravitational wave signal that was found independently by two separate matched filtering methods. This is a crucial cross-check on the initial detection made by the ‘burst’ check, and verifies that LIGO did indeed see two black holes collide more than a billion light years away.
Read More:
- The paper itself: First results from the search for binary black hole coalescence with Advanced LIGO.
- The main detection paper: Observation of Gravitational Waves from a Binary Black Hole Merger.
- The main detection paper’s science summary can be found here.
- Parameter estimation science summary.
- LIGO Open Science Center (with access to GW150914 data): https://losc.ligo.org