What are gravitational waves?
Gravitational waves are oscillations in the fabric of spacetime caused by massive concentrations of matter and energy moving with extreme accelerations. One can think of them as analogous to ripples propagating on the surface of a pond after a rock is thrown into the water. Gravitational waves were predicted by Albert Einstein’s theory of general relativity back in 1916, but it took 100 years to finally measure them directly at the Laser Interferometer Gravitational-Wave Observatory (LIGO). They travel through the universe at the speed of light, passing through everything in their path. These waves carry valuable information about the events that produced them.
Processes leading to strong gravitational-wave signals include cataclysmic events such as: black hole mergers, neutron star mergers, black hole-neutron star collisions, and supernovae explosions. Weaker gravitational waves are produced by single rotating neutron stars containing nonuniformities near their surface, or waves emitted in the early Universe through first-order phase transitions and other phenomena. Other sources of gravitational-waves are discussed below.
The twin LIGO detectors (later joined by it partners Virgo and KAGRA) have been searching for gravitational waves since 2002. The first detection of a signal was observed in September 2015 and reported in February 2016. That event initiated a renaissance in the field of gravitational wave physics and astronomy. Since then, numerous detections have been made, mostly involving signals from merging black holes, but several of them involve neutron stars as well. For more information see the history of LIGO and details on the LIGO detections.
How do we detect gravitational waves?
Gravitational waves cause spacetime to alternately stretch along one direction and compress in the perpendicular direction. Since typical sources are situated far away from Earth, this effect is extremely small, and one needs to measure it using a sophisticated device called an interferometer, which is the mechanism behind LIGO and other gravitational wave detectors. An interferometer consists of two 2.5 mile-long vacuum tubes (or “arms”) arranged in an “L” configuration and with mirrors at both ends. A laser beam is split at the corner of the L and bounces back and forth between the mirrors in each arm. After returning to the beam splitter, the two laser beams undergo interference and resulting intensity is recorded by a photodetector. When no gravitational wave passes through the interferometer, the two beams interfere destructively and the light intensity at the photodetector is zero (up to noise-induced fluctuations). However, the passage of a gravitational wave changes the light travel time up and down one arm relative to the travel time in the perpendicular arm. This relative time delay results in constructive interference when light from the two arms is combined, causing an increase in the light intensity at the photodetector. The oscillating gravitational wave stretches and compress the distance light travels in each arm, and this produces a fluctuating intensity in the measured light output.
The effect of a typical gravitational wave on the LIGO mirrors is truly miniscule – it causes oscillations of the relative length of the arms of the interferometer that are ten thousand times smaller than the width of a proton! Measuring length to such a great accuracy is equivalent to determining the distance to the nearest star (beyond our Sun) to within the width of a human hair. The resulting interference pattern provides information about the origin of a given gravitational-wave signal, including the masses and motions of the objects involved in the event.
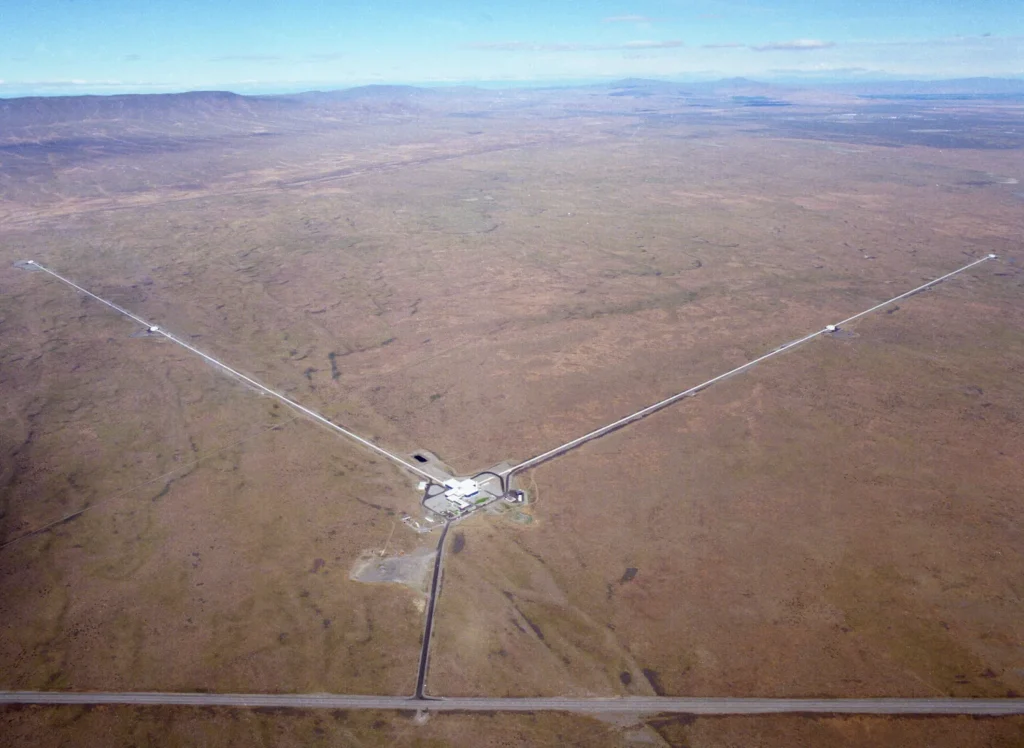
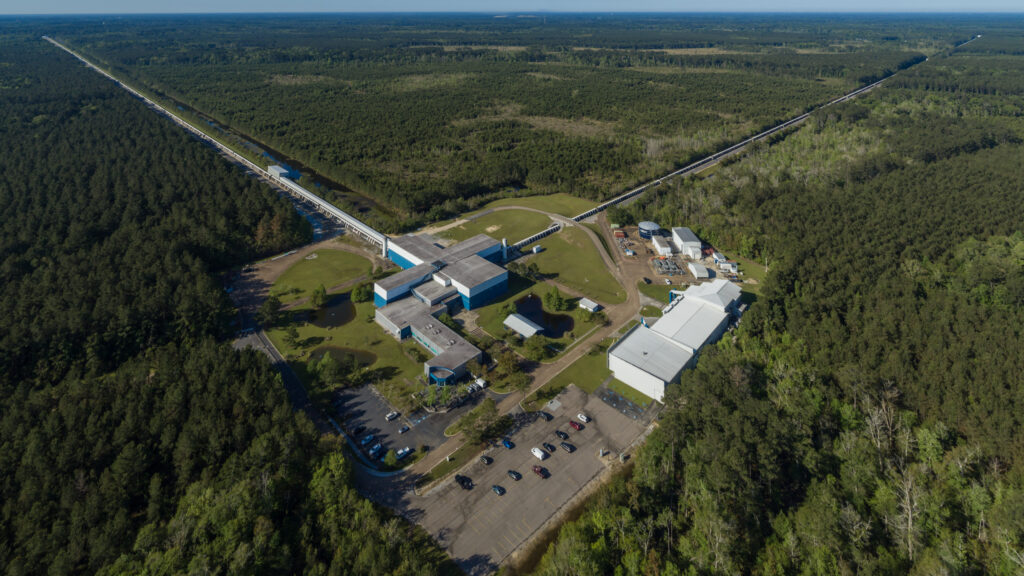
While strong signals can be observed in a single detector, recording a gravitational-wave event in more than one detector increases confidence that the signal is from a real astrophysical event and not from detector or terrestrial noise. LIGO consists of two interferometers – one located in Hanford, Washington and the other in Livingston, Louisiana. Information from our international partners (Virgo and KAGRA) can further increase our detection confidence. Using the signal time-delay and amplitudes measured in multiple detectors also allows us to constrain the direction on the sky from which the gravitational wave signal originated.
The LIGO detectors make use of a range of sophisticated technologies, including highly stabilized lasers, active and passive vibration reduction, ultra-high vacuum, quantum squeezing, and state-of-the-art optical coatings. To learn more about the technology that goes into the LIGO detectors, check out the links below:
- LIGO Lab Technology
- Looking Deeper into LIGO Technology
- LIGO’s Impact on Science and Technology
- LIGO R&D
- Advanced LIGO page at MIT (esp. Technology Development & Migration)
What produces gravitational waves?
One can distinguish four main source classes of gravitational waves based on characteristics the signal (such as its duration and frequency spectrum): inspirals, continuous waves, burst sources, and stochastic sources.
Inspirals
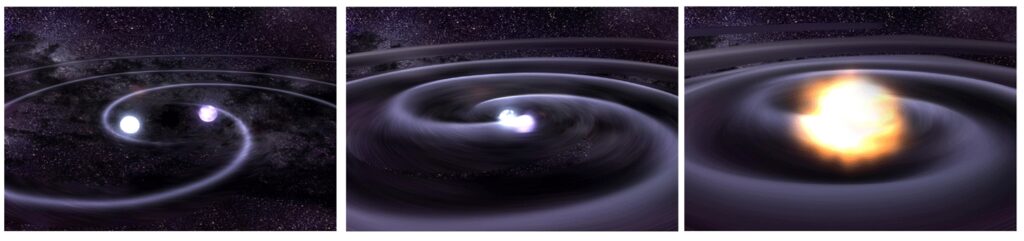
These are the only types of sources currently observed by ground-based gravitational-wave detectors. Inspiral gravitational waves are generated during the final stages of life of compact binary systems. Such systems usually consist of orbiting pairs of two black holes, two neutron stars, or a black hole and a neutron star. When these compact objects are in sufficiently small orbits, the resulting gravitational-wave emission further shrinks the orbits until the two ojects merge or coalesce. Hence, inspiral events are sometimes referred to as “compact binary coalescences.”
Black holes are objects whose gravity is so strong that nothing, not even light, can escape from them. They are typically formed when massive stars collapse at the end of their life cycle. LIGO and other ground-based detectors can detect black holes with masses smaller than about 200 solar masses. However, much heavier “supermassive” black holes can form from different processes and are believed to inhabit the centers of nearly every galaxy. Neutron stars are also formed from the collapsed cores of massive stars, but they are not completely dark. They are primarily composed of neutrons and, if they are fast spinning, can emit electromagnetic radiation and become pulsars.
As the distance between the two components of a binary system decreases and their relative orbital speed increases, the frequency of the resulting gravitational wave increases to its maximal value at coalescence. When translated to sound waves, these frequencies produce a “chirp” sound.
Continuous waves
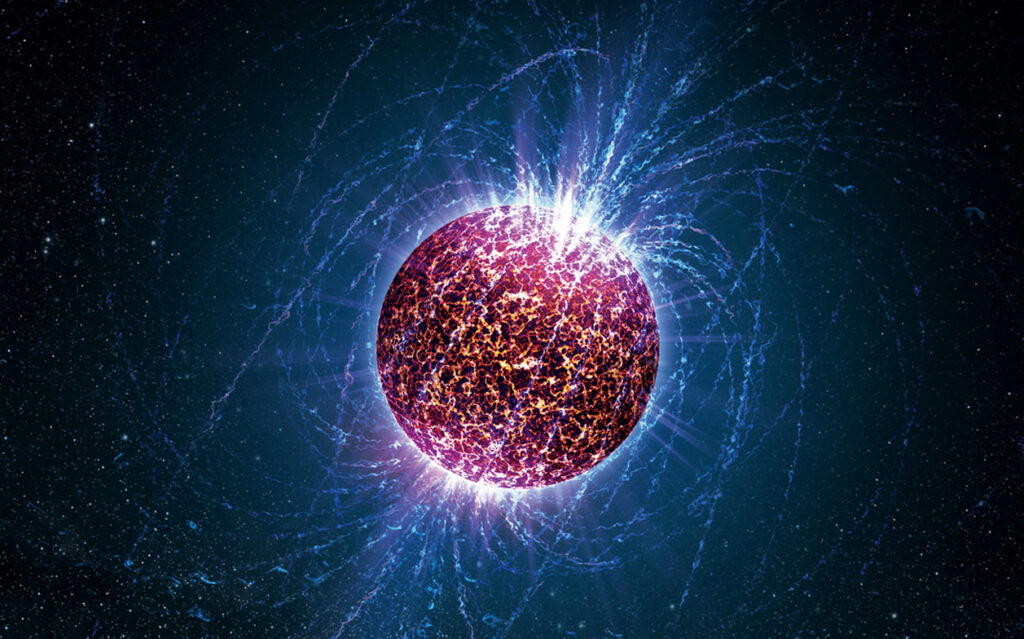
Continuous gravitational waves are produced by systems that exhibit a nearly constant oscillatory behavior. Because these long-lived signals do not change significantly over the time of observation, they are effectively continuous. There are two main sources of such signals: (i) binary systems of compact objects orbiting each other long before they merge, or (ii) individual rapidly rotating neutron stars (not in binaries) with that are elliptically distorted or have other near-surface nonuniformities. The former have frequencies too low to be detected by LIGO or other ground-based interferometers.
Continuous wave sources are expected to produce relatively weak gravitational waves. The frequency of these waves is roughly constant and would correspond to a continuous humming tone when translated into sound. For LIGO, the most relevant sources of continuous gravitational waves are individual neutron stars in the Milky Way. Gravitational waves from these objects have not yet been discovered. But once sufficient detector sensitivity is reached, we may observe them continuously for months or even years.
Burst sources
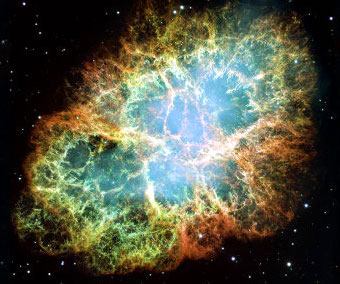
“Burst” gravitational waves are short-duration signals. Events such as supernovae explosions or certain classes of gamma ray bursts may produce burst gravitational waves. In addition to their short duration, these signals may contain complex features that are difficult to model, arising from the complex nature of the explosion dynamics. Burst signals may also arise from unknown or unanticipated sources. While techniques for finding burst signals have been applied to detect the final stages of a binary inspiral and merger, a true burst source has yet to be detected. The search for burst gravitational waves is truly a search for the unexpected.
Stochastic
A stochastic gravitational signal refers to a combination of many weak gravitational waves that are constantly passing through the detector from all directions in the sky. Because the individual distant sources producing these waves are not related to each other, the combined signal has a random noise-like pattern that cannot be precisely predicted but may be analyzed statistically. While a stochasic signal has not yet been seen by LIGO, the key to uncovering these signals is to search for similarities in the noise between multiple detectors.
One possible source for a stochastic signal are the weak unresolved compact object mergers happening throughout the Universe. A more exciting possibility is a stochastic signal that could have been produced in the very early Universe. Detecting those relic gravitational waves would allow us to observe aspects of the Universe a small fraction of a second after the Big Bang, farther back in the history of the Universe than any previous exploration. Such a stochastic gravitational wave background could arise from various phenomena, including first order phase transitions, inflation, or the dynamics of cosmic strings and domain walls. Any discovery of this type would expand our understanding of the fundamental laws of physics.
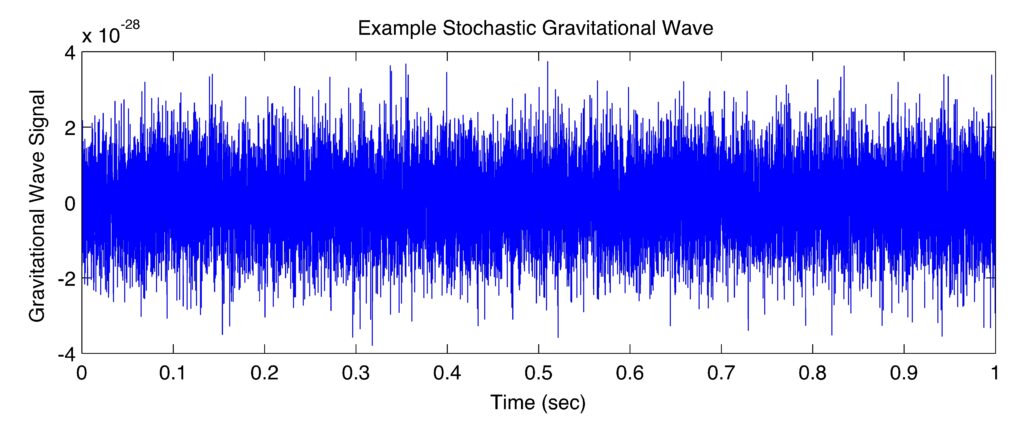
The search for stochastic gravitational waves at LIGO is complementary to searches at lower frequencies conducted by pulsar timing array experiments such as the International Pulsar Timing Array (IPTA). Those efforts rely on searching for correlations in the arrival times of radio signals produced by pulsars at different positions on the sky. A stochastic gravitational-wave signal will change the distances between those puslars and the Earth, leaving an imprint on the pulse arrival times. In 2023, evidence of such a signal was announced by the North American Nanohertz Observatory for Gravitational Waves (NANOGrav), the European Pulsar Timing Array (EPTA), the Parkes Pulsar Timing array (PPTA), and the Chinese Pulsar Timing Array (CPTA). The signal is consistent with expectations for a stochastic gravitational-wave background from merging supermassive black holes, however other sources for the stochastic signal have also been suggested.
Challenges
The LIGO detectors are true masterpieces of engineering, uniquely designed to measure the tiny effects of passing gravitational waves on the interferometer arms. To achieve their exquisite precision, multiple experimental challenges must be overcome. These include the effects of ground vibration, light scattering within the beam tubes, noise associated with the laser and the resulting photons impinging on the mirrors, thermal noise in the mirror and its suspensions, and electronics noise. Mitigating these noise sources requires multiple feats of engineering. For example, the 4 km-long arms sustain the second largest ultra-high vacuum chamber in the world, keeping a volume of 354 thousand cubic feet at one-billionth of the Earth’s atmospheric pressure. The LIGO mirrors are mounted on sophisticated seismic isolation systems that damp ground vibrations. And the pair of detectors themselves (along with international partners like Virgo and KAGRA) allow us to sift real signals from the noise by looking for near-simultaneous signals in both detectors. Learn more about the technology of LIGO at the LIGO lab website.
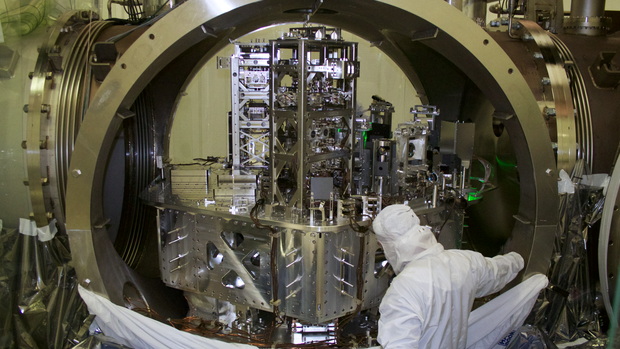
Detecting gravitational waves also involves overcoming theoretical and computational challenges. A precise mathematical model for the gravitational-wave signal (in the absence of noise) must be constructed; this requires solving the Einstein field equations, a highly complex and nonlinear set of partial differential equations. These are solved via a combination of analytical techniques and numerical simulations. Detection then requires evaluating the signal model for a very large number of model parameter values (e.g., possible combinations of black hole masses and spins), searching the data stream to determine if a close match to a particular signal model is actually present in the data. Once a potential signal is found in the data, another computationally intensive process (parameter estimation) involves refining the parameters of the model that describes the source of the gravitational waves (e.g., a binary black hole or a neutron star/black hole pair).
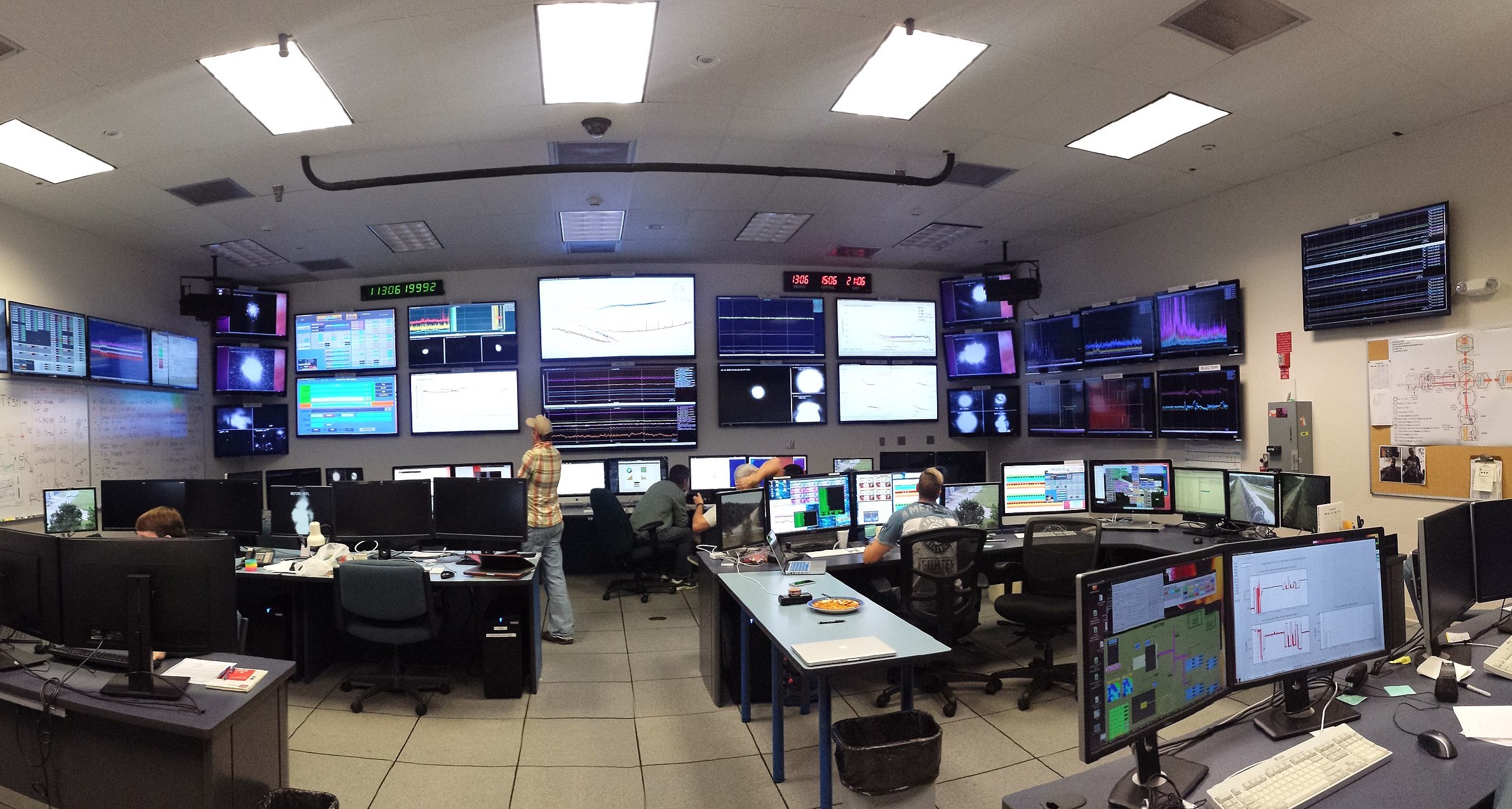
What do we hope to learn?
The detection of gravitational waves by LIGO has initiated an extremely exciting period in modern physics and astronomy. The vast majority of prior astronomical observations have relied on electromagnetic (EM) waves (e.g., visible light, radio waves, gamma rays). But EM waves are easily absorbed or scattered by any matter between the source and us. In contrast, gravitational waves pass through all matter with very minimal absorption or scattering. This allows gravitational-waves to reveal very distant objects and to peer inside the sources that produced those waves. The details of the waves (their frequencies and amplitudes) encode information about their sources (e.g., the masses, spins, distances, and other properties).
Some of the most intriguing astrophysical questions that LIGO is addressing include: How do black holes form? How do they get their masses and spins based on their formation environment? Is general relativity the correct description of gravity? How does matter behave under the extreme temperatures and pressures inside neutron stars? What exactly happens during a supernovae explosion?
As mentioned above, gravitational waves may also be the key to understanding some of the most fundamental questions about our universe: What happened within the first one-trillionth of a second after the Big Bang? An observation of a primordial stochastic gravitational wave background would let us peek into the earliest stage our universe’s evolution, perhaps offering insight into new physics at high energy scales, the nature of dark matter, or the origins of dark energy.
What’s next?
Currently LIGO is conducting its fourth observing run. The full timeline of past observing runs along with the plan for upcoming observations can be found at LIGO, Virgo, KAGRA observing plan website. With increasing sensitivity and more accumulated data, larger numbers of compact binary mergers are expected to be observed. The chances of discovering continuous, burst, or stochastic gravitational wave signals will also significantly improve. A third LIGO detector in India (the LIGO India project) is also underconstruction and will significantly improve both our search sensitivity and our ability to determine the direction from which gravitational wave signals originate.
The community of gravitational-wave scientists is also developing plans for future gravitational wave detectors, both ground-based and space-based. Some of these planned future experiments include:
Learn more
For more information on LIGO and the science it explores visit: