Background
Neutron stars are dense, neutron-rich stars left behind by the aftermath of a supernova explosion. They usually weigh a little bit more than the sun, but are only about 10-20 kilometers across. A small percentage of these neutron stars have an exceptionally strong magnetic field — about a trillion times that of the sun — and we call these “magnetars”. Figure 1 shows an artist’s rendition of a magnetar.
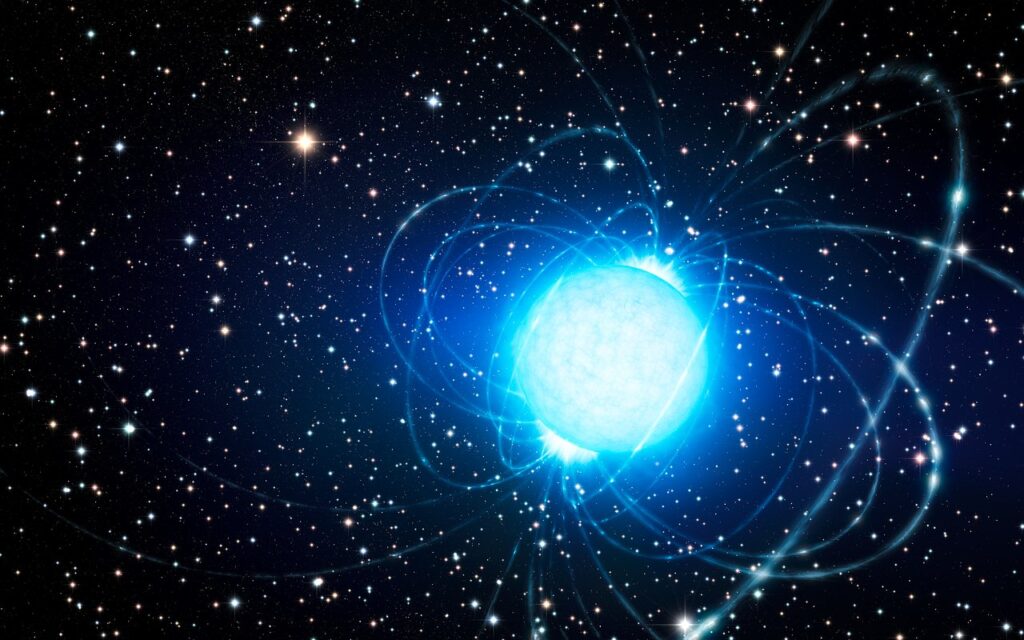
Figure 1: An artist’s rendition of a magnetar. (Credit: ESO/L. Calçada (CC BY 4.0))
There are only 30 known magnetars, and 28 of them are in our galaxy. We think that there are more in distant galaxies, but it is more difficult to discover them because of the limitations of our instruments. Each of these magnetars sends out “X-ray bursts”, which are blasts of light in the X-ray frequency range, but the properties of each of these bursts varies wildly and depends on the magnetar emitting it. These bursts tend to come in batches, so a magnetar might emit tens of bursts over the space of days to weeks, and then it might go quiet for a period of time, even up to several years. We observe these bursts through X-ray telescopes mounted on satellites. Because of the large distance to the magnetars, the X-rays that reach us here on Earth are so weak that they pose no threat to us
The causes of these bursts are still an open mystery, but there have been several observational clues that have made this mystery more tantalizing in recent years. On April 28th, 2020, one of the X-ray bursts was accompanied by a fast radio burst, which is a short and highly energetic burst of light in radio frequencies. The causes of fast radio bursts are another astrophysical mystery, but the association between these two phenomena does give us some clues as to what to look for in the future. Another interesting development was the discovery of “periodic windowed behavior” — periodically repeating segments of time in which a magnetar does not burst — in one of the magnetars in our study. It still remains unknown whether all magnetars display periodic windowed behavior, but it has been established that some repeating fast radio bursts do.
The X-ray telescopes that we use for detecting X-ray bursts don’t give us any information on what actually happens in the magnetar to cause the burst. This is where gravitational waves (GWs) come in. GWs are vibrations in space-time that travel through the universe at the speed of light. They are created by lumps of matter which are moving quickly. The most famous GW sources are pairs of black holes orbiting each other, however, it could very well be that a magnetar becomes momentarily deformed such that it also contains the necessary properties to generate GWs. If we were to detect a GW associated with a bursting magnetar, then we would be able to analyze its properties and take a guess at what was happening inside the magnetar at the time which could have caused both the GW, and the X-ray flare. If we don’t detect one, we can estimate a threshold amplitude above which we know that we would have seen a GW signal if one were present. In either case, we learn something new about magnetars, which will help in unraveling the mystery of their bursts.
There were 16 bursts from 3 different magnetars during the observation time that were considered in this paper, and the goal is to search for GWs accompanying them.
Searching for Gravitational Waves
GWs shrink and expand space-time in a certain direction. So if a GW were to hit you from the front, it would make you taller and thinner, and then shorter and wider, and then taller and thinner, and so on. But for typical distances to the GW source, your height would only change by about a billionth the radius of an atom! We detect these GWs by using lasers to monitor the motion of suspended mirrors at the two LIGO observatories located in Louisiana and Washington, USA, and the Virgo observatory located in Cascina, Italy.
In the case of magnetars, we already know exactly when and where the X-ray burst happened from the X-ray telescopes’ data. So we can combine the data from the LIGO and Virgo observatories such that we only focus on the direction in the sky where the magnetar is, and just around the time of the burst. The difficult part is trying to estimate the significance of any possible GW signal at the time of the X-ray burst. To make this estimation we compare it with data from other times when we know the magnetar was not producing X-ray bursts (and presumably not GWs).
Theoretical models give us clues that there are two types of GWs that might be produced by these X-ray bursts. GWs of the first type are short-duration (milliseconds) and high-frequency (1–3 kHz), and likely produced by a quick displacement in the magnetar’s core. The second type of GWs are longer duration (tens to hundreds of seconds), and range in frequency from 20Hz to 2000Hz. We searched for signals resembling these using two different software analysis packages, but each search is minimally modeled, meaning that they still have some capability of detecting a signal even if that signal does not match precisely with the theoretical predictions.
Results and Future Prospects
Neither type of GW was detected. In order to determine the sensitivity of our analysis, we added artificial data into the real data at the time of the X-ray bursts. This artificial data represents a simulated GW of plausible properties, and then we noted at which amplitude such simulated GWs become detectable by the LIGO/Virgo detectors. We also were able to calculate the GW energy that corresponds to these detection threshold amplitudes, and also the ratio of the GW energy to the electromagnetic energy. These upper limits are displayed in Figure 2.
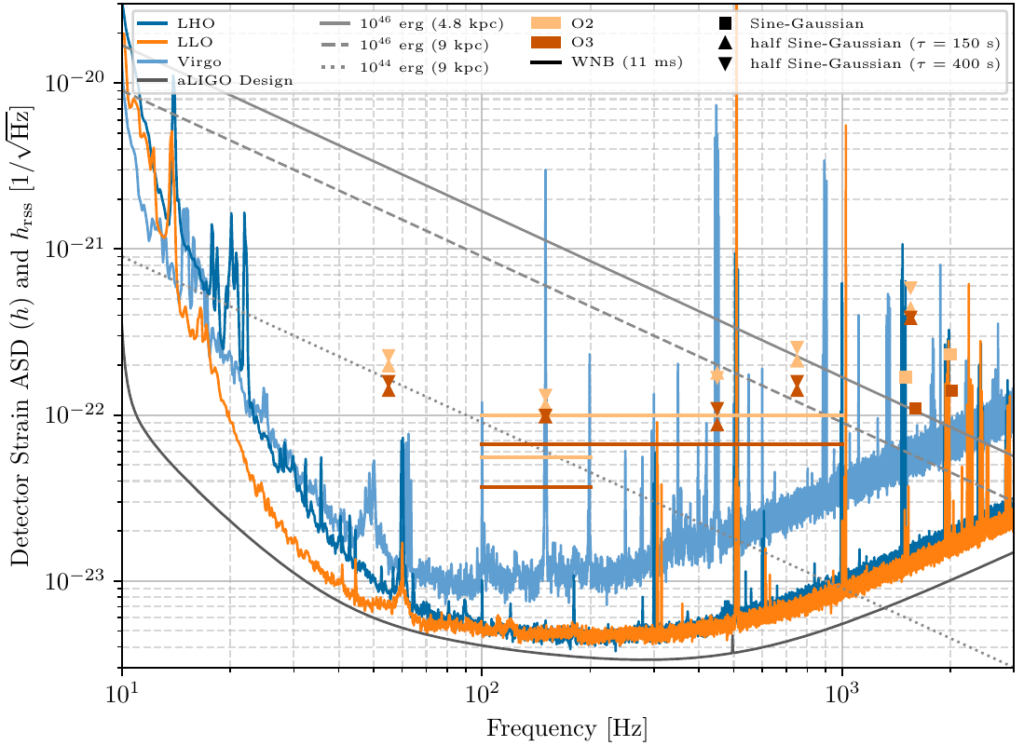
Figure 2: The upper limits for detecting our simulated data are shown for the third observing run, and the second observing run, and both are compared to the detector strain amplitude. This is the 5th figure displayed in the paper.
The sensitivity of the detectors is frequency-dependent, and the frequency is represented on the horizontal axis of Figure 2. The vertical axis displays the strain in the detector due to background noise at each frequency, and the lines marked “LLO”, “LHO” and “Virgo” indicate each detector. You can see that these have many prominent spikes — these are frequencies where the background noise couples particularly well to the strain. We added artificial data with two different shapes (sine Gaussian and half sine Gaussian), and the triangles and squares on the graph represent the strain at which these signals would have been detected. We see some improvement over the second observing run at all frequencies, and our sensitivity for some simulated GWs improves by almost a factor of 2! Our search for long duration GWs has the best upper limits in the history of all searches for long duration GWs from bursting magnetars.
Even though we obtained threshold values for the GW amplitude and energy that we could detect, these limits were not low enough to be enlightening. All of the current models that attempt to explain magnetar X-ray flares also give predictions for the energy of the accompanying GW and these energies are lower than our upper limits. So our upper limits were greater than they would need to be in order to expand our understanding of the internal dynamics of a magnetar.
We are looking forward to expanding on our success as GW astrophysics advances as a field. All of the observatories are undergoing upgrades that will further enhance their sensitivity, and allow us to see farther out into space. The 4th observing run will also be joined by the KAGRA detector in Japan, which will increase the network sensitivity in more sky directions. We are also making preparations for a stacked analysis — in which we examine all of the magnetar X-ray flares at the same time in order to increase the sensitivity of the search. These upgrades and new analysis tools will hopefully help us to understand the internal dynamics of magnetars, and the extreme physical environments they contain.
Find out more
- Visit our websites:
- Read a free preprint of the full scientific article here or on arXiv.org