On January 5th 2020 the Advanced LIGO detector in Livingston, Louisiana in the US and the Advanced Virgo detector in Italy observed gravitational waves consistent with an entirely new type of astronomical system. The gravitational waves were produced by the death spiral of two of the most extreme objects in the Universe: one, a neutron star, and the other a black hole. LIGO and Virgo observed the last few decaying orbits (inspiral), followed by the merger of the neutron star with the black hole. Remarkably, just ten days later a second gravitational-wave signal from the inspiral and merger of a neutron star with a black hole, was observed, this time by both Advanced LIGO detectors (in Livingston and also in Hanford, Washington) and the Virgo detector. This is the first time gravitational waves have been observed from a mix of neutron stars and black holes (see Figure 1). Before this new discovery, gravitational waves had been observed from the merger of pairs of black holes and pairs of neutron stars. Our new discoveries are nick-named GW200105 and GW200115.
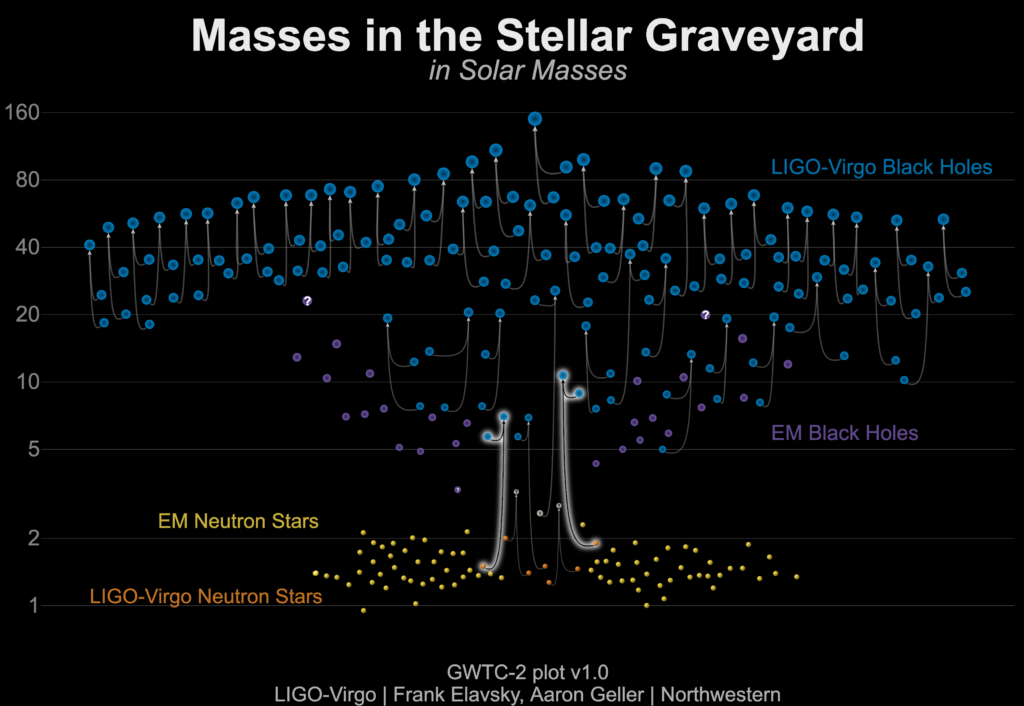
Figure 1: The masses of neutron stars and black holes measured through gravitational waves and electromagnetic observations. The yellow and purple markers represent the electromagnetic measurements of neutron stars and black holes, respectively, while the orange and blue markers are the corresponding measurements using gravitational waves. Our signals, GW200105 and GW200115, are highlighted as the merger of neutron stars with black holes. (Image credit: LIGO-Virgo & Frank Elavsky, Aaron Geller, Northwestern University)
These two discoveries represent the first detections of neutron star-black hole (NSBH) systems. For several decades, NSBH systems had been predicted to exist, but without convincing observational evidence until now. With the observation of NSBHs, we now have seen all three types of binaries that can be formed from black holes and neutron stars. Systems made of black holes and neutron stars are known as “compact binaries”. These new discoveries, together with future observations of compact binaries, will shed light on the birth, life, and death of stars, as well as the surroundings in which they form.
Detecting the gravitational-wave signals
The search for gravitational-wave signals in the data recorded by the detectors uses “matched filtering”. This compares the observed, noisy, data to predictions of signals from Einstein’s General Relativity. Matched filtering can pick out gravitational-wave signals from noisy data in a way similar to how we can distinguish individual instruments in a piece of music. We consider GW200115 to be an astrophysical gravitational wave signal with very high confidence, with a chance of occurring due to random noise of less than once in 100,000 years. The astrophysical nature of GW200105 is more challenging to establish statistically, but it also clearly stands apart from all noise effects we’ve ever seen, and we consider such a signal likely to occur due to noise less than once in 2.8 years.
NSBH mergers can, in principle, produce light across the electromagnetic spectrum. Unfortunately, the sky direction of the sources could be measured only very imprecisely, to an area of sky between 2,400-29,000 times the size of the full moon. Together with the large distance to the source (discussed in more detail below), this made observation of electromagnetic light unlikely, and none was observed. Future observations of NSBH mergers may produce observable light which could possibly reveal the black hole “tidally disrupting” (tearing apart) the neutron star. This could provide information about the extreme form of matter that makes up neutron stars.
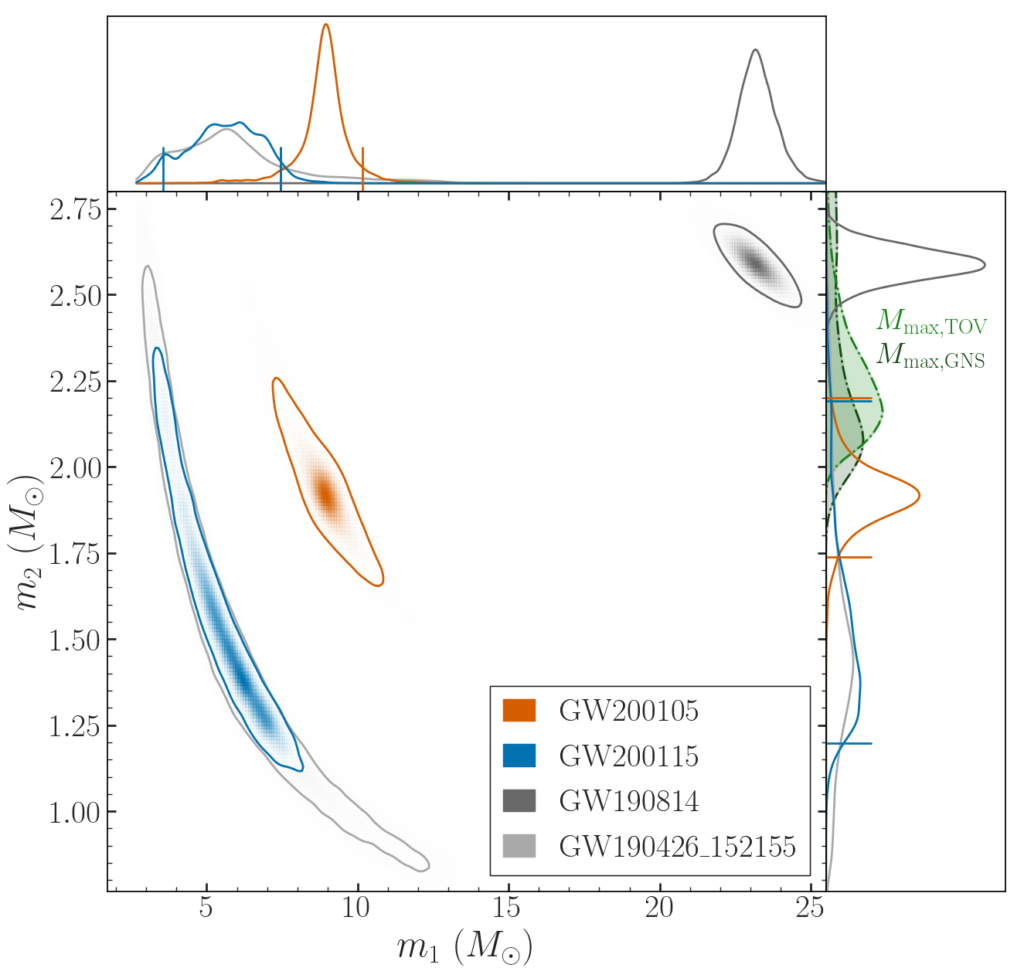
Figure 2: (figure 4 from the publication) Summary of our knowledge of the component masses of the objects that produced GW200105 and GW200115. The horizontal axis represents the mass of the heavier object (the black hole), whereas the vertical axis represents the mass of the lighter object (the neutron star). The color-shading indicates mass-combinations consistent with the data, orange for the first event, and blue for the second event. Darker shading indicates better agreement, i.e. a higher probability for such mass-combinations. The top panel summarizes the information about the black hole mass, with — for instance — the blue curve showing that the black hole in GW200115 had a mass somewhere between ~3.5M☉ and ~7.5M☉. The right panel summarizes the information about the neutron star mass — for instance, the orange curve in this panel indicates that GW200105’s neutron star had a mass between 1.75M☉ and 2.2M☉. The green shadings in this right panel summarize the present astronomical knowledge about how massive neutron stars can be, showing that our observed objects have masses small enough to be neutron stars. The figure also shows information about two earlier gravitational wave discoveries: GW190814, which is probably the merger of a 23 M☉ black hole with a 2.5 M☉ black hole (the lightest ever observed); and GW190426_152155, a signal that looks like a NS-BH system, but is so weak that it is unclear whether it is of astrophysical origin.
Properties of the sources
Gravitational waves encode rich information about their origin, for example, the masses of the compact binary stars. The black hole and neutron star that created GW200105 are about 8.9 times and 1.9 times as massive as our Sun (M☉), respectively. The GW200105 event happened around 900 million years ago, hundreds of millions of years before the first dinosaurs appeared on Earth. For the GW200115 event, we estimate the black hole and neutron star had masses around 5.7 M☉ and 1.5 M☉ respectively, and their merger happened almost 1 billion years ago. The masses are summarized in Figure 2. We found the black hole spin for GW200105 could lie between 0 and as high as 30% of the maximum rotation rate of black holes, while for GW200115, the spin lies between 0 and 80% of the maximum rate. We do not have strong evidence of neutron star spin because our measurements are not sensitive to it (see Figure 3).
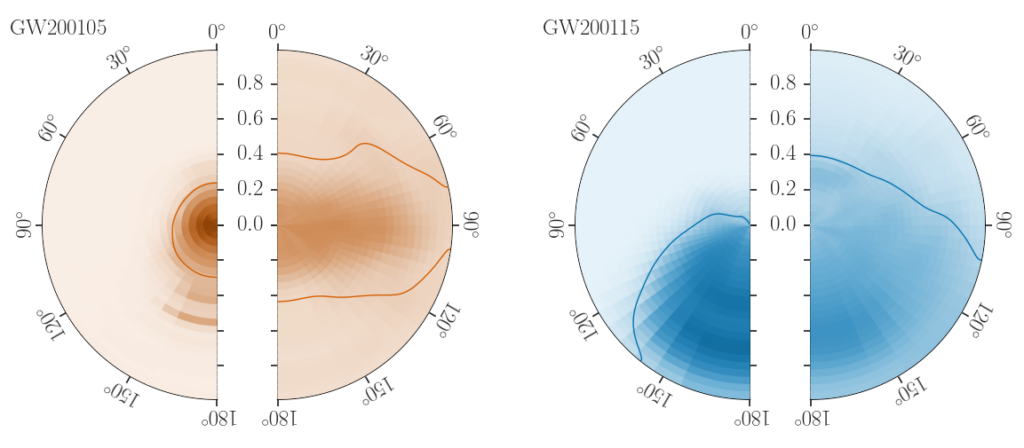
Figure 3: (figure 6 from the publication) The inferred spin magnitude and direction of the black holes (left half-disks) and neutron stars (right half-disks) of GW200105 and GW200115. The radius of the disk indicates the spin magnitude, and ranges between 0 (no spin) to 1 (maximum rotation rate of black holes). The spin direction is shown as an angle, which ranges from 0o (objects spin in the same direction as the orbit of the binary) to 180o (objects spin in the opposite direction to the orbit of the binary). Shading indicates probable values of spin magnitude and direction. The left-most half-disk has shading that peaks near the centre, indicating that GW200105’s black hole has a spin that is likely small. The second to right half-disk’s shading extends downward, indicating that GW200115’s black hole may be spinning in a direction opposite to the orbital motion.
Why do we think we observed NSBHs? To produce the observed gravitational waves, the objects have to be very compact and dense compared to typical stars, since otherwise they would break apart before they merged. The masses of the heavier objects in each binary are 8.9 M☉ and 5.7 M☉ so the only known objects they could be are black holes. The masses of the lighter objects are around 1.9 M☉ and 1.5 M☉, much lighter than any known black hole. These masses are consistent with known neutron stars, like the ones observed in the Milky Way, or with gravitational waves (e.g., GW170817). The masses of the black holes are consistent with predictions from models of star formation and evolution.
How did they form, and how often does this happen?
So, how did these NSBH systems form? There are two main possibilities. One of these starts with two stars already orbiting each other. The stars have masses such that when they age, they eventually explode in supernova explosions, one star leaving behind a black hole and the other leaving a neutron star. This is called “isolated binary evolution”. The other possibility is that the neutron star and black holes form from separate stars in unrelated supernova explosions, and only afterwards find each other. This is called “dynamical interaction” and can occur in dense stellar environments such as globular clusters. To distinguish between these two possibilities, the spin orientations of the black holes give a strong hint. In isolated binary evolution, the spin directions of black holes tend to align with their binary orbit, i.e., we expect the neutron star to orbit in the equatorial plane of the black hole. In contrast, the dynamical interaction scenario doesn’t prefer a particular direction of the spin, and so the neutron-star orbit could have any orientation relative to the black hole’s equatorial plane.
The inferred black hole spin of GW200105 does not allow us to distinguish either of these formation scenarios. However, for GW200115, we find that the black hole spin direction is likely to be opposite to the direction of the binary orbit. For example, if the neutron star orbits the black hole clockwise, then the black hole would rotate on its axis counterclockwise. This hints that the source of GW200115 could have formed in a dense environment, such as a globular cluster.
How many NSBHs in the Universe merge within a given time period? The observation of the two NSBH systems tells us that between 5 and 15 such systems merge per year within a distance of one billion light years. Also, this estimated merger rate could be explained by isolated binary evolution or dynamical interaction in young star clusters alone but we cannot single out a specific scenario.
Find out more:
- Visit our websites:
- Read the full scientific article in “Astrophysical Journal Letters” or a free preprint here.
- Read the LIGO and Virgo press releases about this discovery:
- Have a look at
- the detection page with additional multimedia and resources for these discoveries
- a summary fact sheet for these discoveries [translations available here]