Short introduction
Stellar collapses are among the most energetic astrophysical phenomena. They happen when a massive star whose mass is larger than ~8 times the mass of the Sun is reaching the end of its lifecycle. Hydrogen has fused into helium, then helium fuses into carbon, etc. The nuclear fusion reactions produce a gas of electrons whose pressure, due to the Pauli exclusion principle, opposes gravity. The temperature and the density of the star increase as the core contracts until it is mainly composed of iron. When the mass of the core exceeds the Chandrasekhar limit of 1.4 times the mass of the sun (denoted M⊙), degenerate electron pressure is no longer strong enough to counter gravity and the core collapses in a fraction of a second. Neutrons and neutrinos are produced. When the density reaches the density of a nucleus the collapse stops and infalling matter bounces, generating a shock wave that eventually stalls. When revived, thanks to the neutrino’s heating, matter convection and/or standing accretion shock instability (SASI), the shock wave expels the outer layers of the star generating the supernova observed in optical telescopes. This particular type of supernova is called core collapse supernova (CCSN).
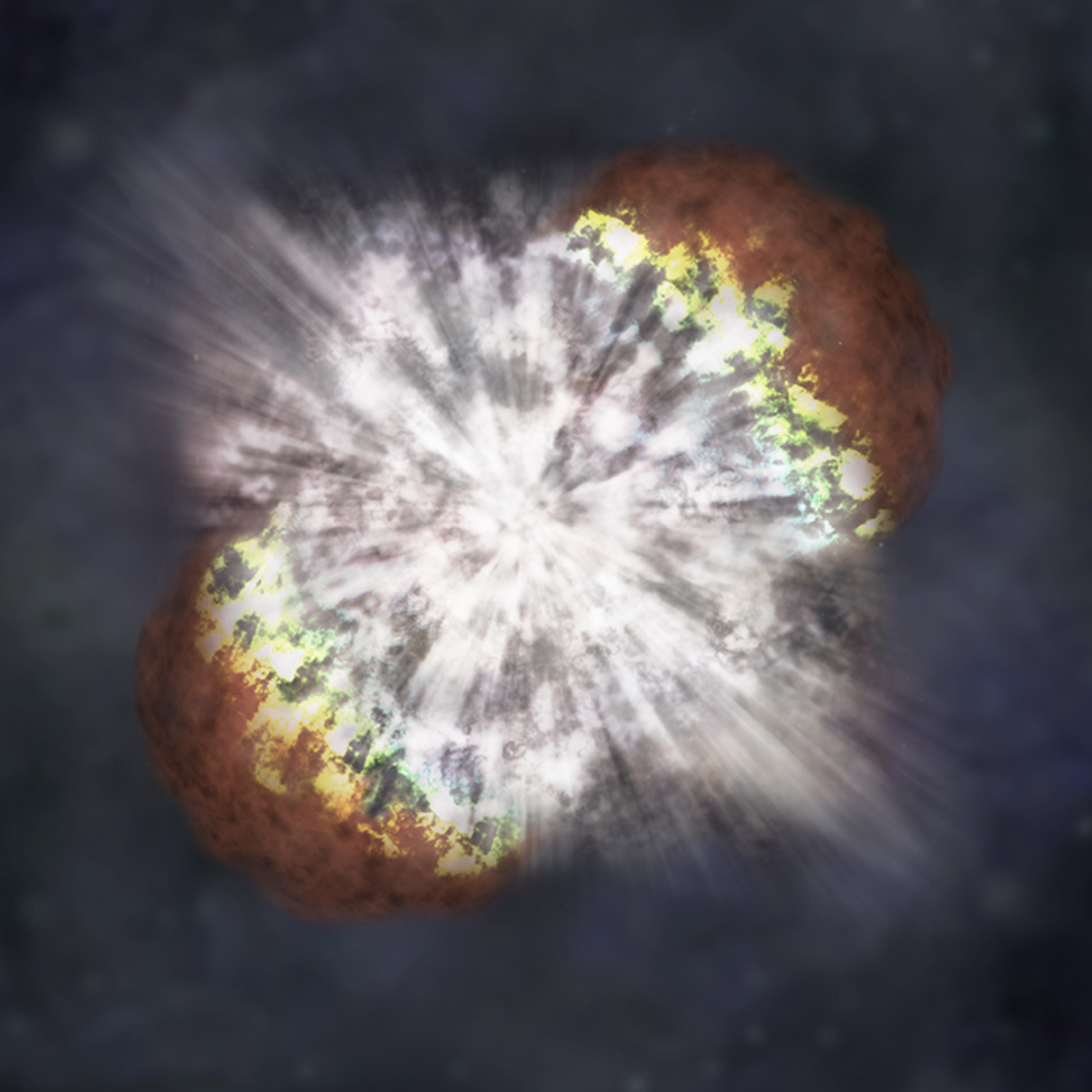
Figure 1: Artistic illustration of a supernova, one of the most energetic phenomena in the Universe that is the fate of massive stars. Image credit: NASA/CXC/M.Weiss
Stellar collapses have been considered to be a source of gravitational radiation for many years. Because the physical processes at the origin of the collapse are rather complex, the gravitational-wave (GW) emission is hard to predict, and a detection would provide a wealth of information about the dynamics of the collapse. However, the gravitational signal is expected to be weak, orders of magnitude weaker than the signal emitted by the merger of two neutron stars or two black holes. Only nearby CCSN, happening in our Galaxy or in a galaxy of the Local group, are expected to emit enough gravitational radiation to be detected by the current network of ground-based detectors composed of the LIGO detectors in the USA, the Virgo detector in Italy and KAGRA in Japan. Unfortunately the CCSN rate is very low. On average, one expects only a few CCSN per galaxy and per century. SN1987A, observed in the Large Magellanic Cloud (50 kpc or 163,000 ly) remains the most recent CCSN in our local universe. To enlarge the number of potential sources, the LIGO-Virgo-KAGRA (LVK) collaboration is searching for GWs emitted by more distant CCSN, within about 30 million light years, or about 10 times farther than the nearby Andromeda galaxy.
Why is SN2023ixf interesting for gravitational-wave astronomy?
SN2023ixf is a type II supernova that was discovered by the amateur astronomer Koichi Itagaki on 2023 May 19th in the host galaxy Messier 101, also known as the Pinwheel Galaxy. What makes SN2023ixf interesting is the relative closeness of this event. Located at 6.7 Mpc (21.8 millions light years) away, this is one of the nearest CCSN discovered while the LVK detectors were taking data. This offered a great opportunity to search for a possible GW counterpart. SN2023ixf can be compared to SN2017eaw that was discovered in a galaxy at almost the same distance. But, at that time, the two LIGO detectors were 2-3 times less sensitive than in 2023.
When SN2023ixf happened, the two LIGO detectors were operational but still in a phase of tuning before the fourth Observing Run of the LIGO-Virgo-KAGRA collaboration officially started on May 24th. As a consequence, the two LIGO detectors were not constantly observing during the period of time where the SN2023ixf collapse happened.
But how do we know when the collapse happened? SN2023ixf has been followed up by many optical, X-ray and radio telescopes. As a typical CCSN, the light curve shows a rise to a maximum at about five days after the supernova discovery followed by a plateau lasting for about one month (see Figure 2). Because of the wealth of observations, the time of the shock breakout, when the light first appears, is pretty well estimated. Yet, this does not tell when the collapse happened as there is a delay between the collapse and the shock breakout that depends on the nature of the star that collapsed. A large set of M101 pre-discovery imaging observations from ground-based telescopes, Hubble Space Telescope and Spitzer Space Telescope suggest the nature of the SN2023ixf star progenitor to be a dusty and variable red supergiant, with an estimated mass ranging from 8 to 20 solar masses. This large uncertainty on the mass of the progenitor implies that the collapse may have happened up to 5 days before the shock breakout. In this time window, the two LIGO detectors Hanford (H1) and Livngston (L1) were simultaneously taking data only 15% of the total time.
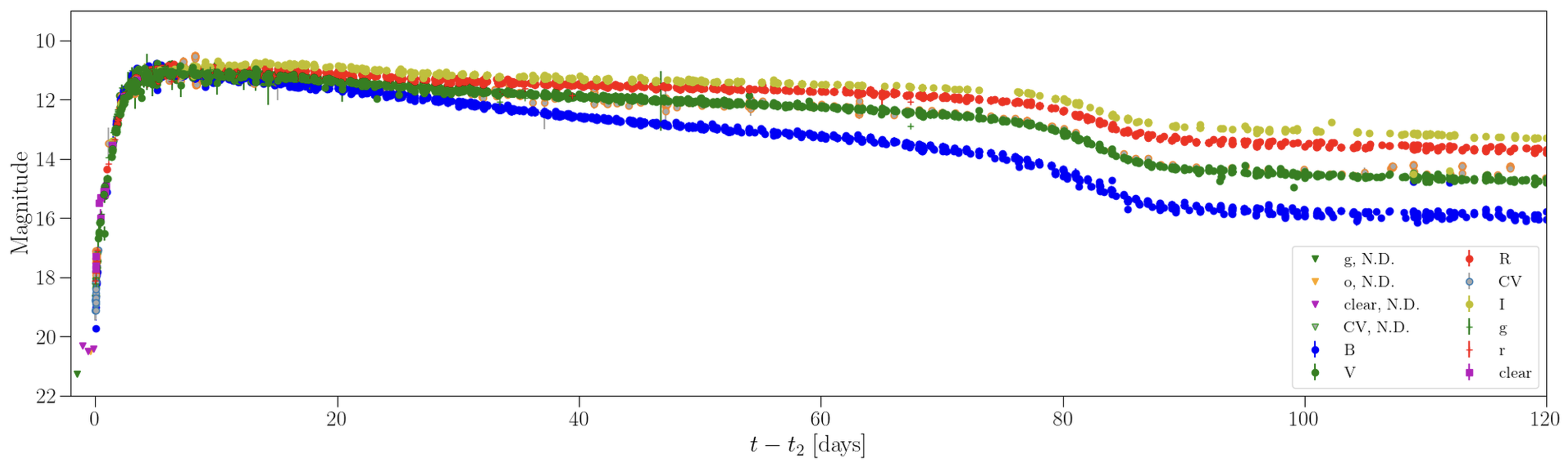
Figure 2: Light evolution of SN 2023ixf covering different photometric bands over 120 days.
What have we found?
CCSN GW signals are complex and can only be predicted by hydrodynamics simulation codes that also consider general relativity as the framework for gravity. The predictions depend on the star progenitor parameters which are unknown. Matched filter techniques are thus not applicable. Instead we rely on the coherent WaveBurst (cWB-XP), an algorithm that searches for correlation in the data of at least two detectors assuming the signal is short – of the order of one second maximum – with frequencies spread between ~20Hz and 2kHz.
No interesting event candidate has been found in the five-days time window where the collapse might have happened. More precisely, the most significant event has a false alarm probability of 76%, making the event likely due to noise. The absence of a GW signal could be due to the fact the collapse happened when the two LIGO detectors were not in observing mode (15% of coverage). The other explanation is the weakness of the emitted GW signal. We have estimated that even the most optimistic models from numerical relativity predict a detectable GW signal only up to the Large Magellanic Cloud located at about 150 thousand light years. This is two orders of magnitude closer than SN2023ixf.
What have we learned?
We have not observed any GW signal associated with SN2023ixf, but if we assume the collapse happened when the two LIGO were in observing mode, we can estimate how energetic the collapse must have been to be detectable with GW detectors. Of course, this depends on the supernova explosion mechanism at play. Was the progenitor star rotating? Was matter convection strong enough to excite the proto-neutron star (PNS) fundamental modes? Was SASI enhancing the neutrino heating? Were magnetic fields playing any role at enhancing the signal? As we do not know the answers, we consider the GW emission of a simple rotating triaxial ellipsoid with an asymmetry (similar to a rugby ball) to model the development of a bar-mode instability that is sometimes present in core collapse simulations. SN2023ixf allows to improve by almost an order of magnitude the constraint on the energy that could have been emitted by this event. For an hypothetical emission at low frequency, we are sensitive to an emission of the order 10−5 M⊙c2 as shown in Figure 3. This quantity corresponds to the energy of a source that would be detected with an efficiency of 50% and for a false alarm probability of 76%. This needs to be compared with the predictions of the CCSN numerical simulations: the range of emitted energy is rather broad from 10−10 M⊙c2 for the less energetic non rotating progenitor to 10−6 M⊙c2 for highly rotating sources, where the energy is expressed in terms of a fraction of the mass of the sun considering the famous Einstein equation E=mc2. In terms of emitted energy, this means with CCSN events such as SN2023ixf we are still several orders of magnitude away from what the less energetic explosion mechanism predicts.
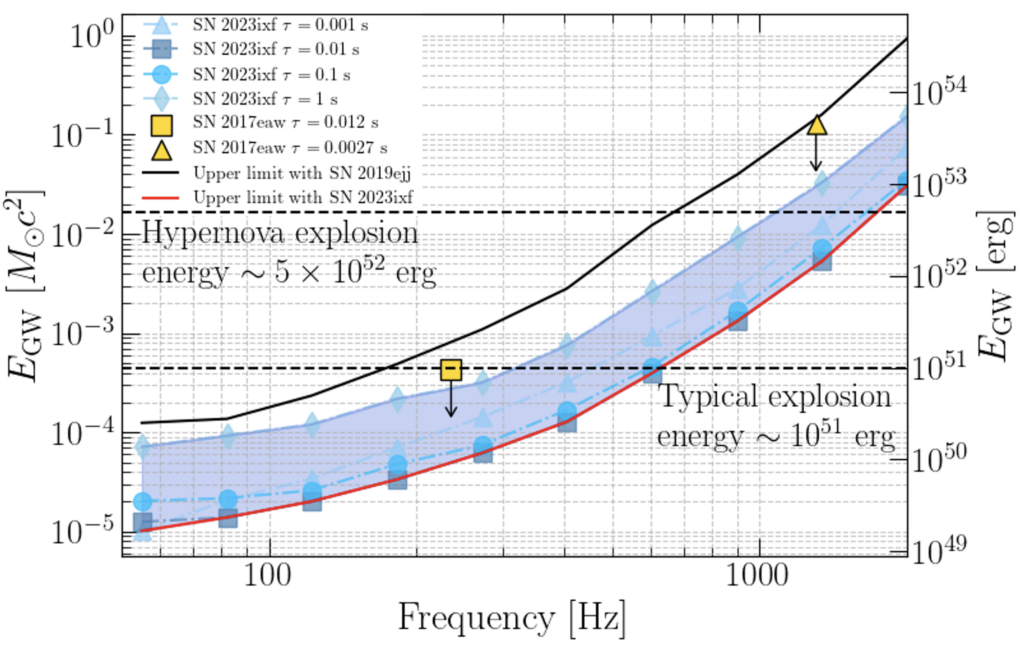
Figure 3: GW energy in units of the solar mass times the speed of light squared, or erg, as a function of the frequency of a bar-mode signal with a detection efficiency of 50% and a false alarm rate of 2.1 per day. The shaded blue region contains results from bar-mode signals of different duration from a few ms up to 1s. The duration of the signal is given by the τ parameter that appears in the legend. The yellow square and triangle markers represent the GW energy that has been constrained using the data for SN2017eaw. The black line shows the best constraint obtained with SN2019ejj. The horizontal dashed lines show the typical electromagnetic energy emitted by a standard supernova and by a hypernova.
The amplitude of the bar-mode GW signal can be parametrized by an asymmetry factor, also called ellipticity. Figure 4 reports the ellipticity for a range of GW signal frequencies and durations. The most stringent constraints on ellipticity are obtained for the longest signals, ranging from 103 at the lowest search frequency to 1.04 at 2 kHz. This improves previous constraints set with SN 2019ejj discovered in the third observing run.
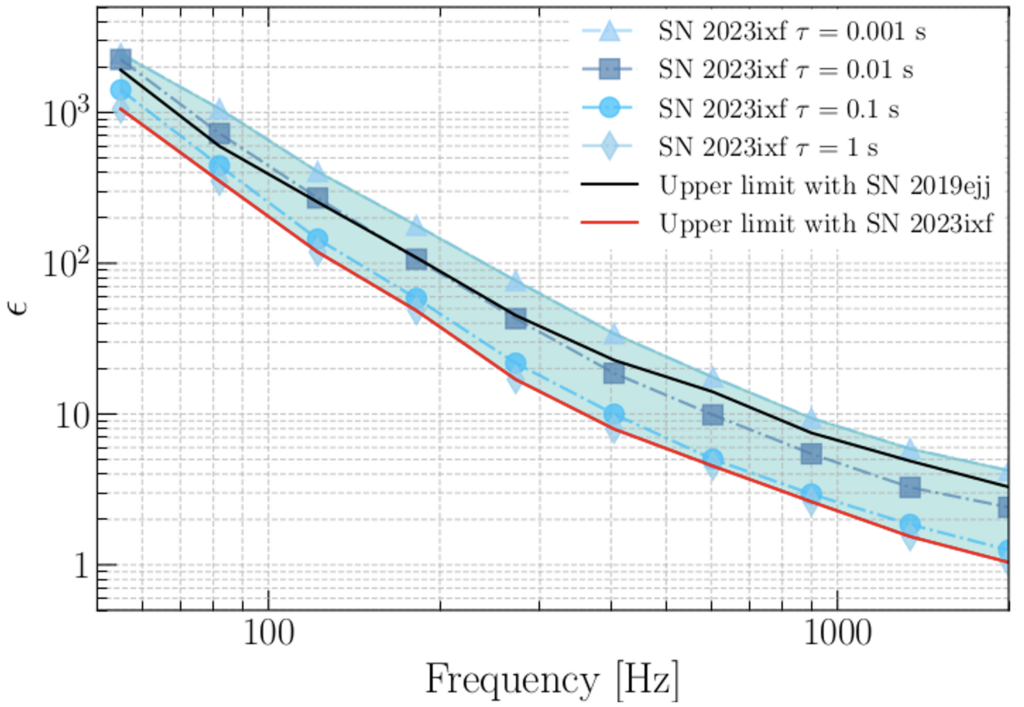
Figure 4: Constraints on the PNS ellipticity as a function of the frequency for bar-mode signals set up by the search for a detection efficiency of 50% and a false alarm rate of 2.1 per day. The shaded region contains results from a wide range of GW signal duration given by the τ parameter in the legend. The ellipticity parameter tells how much the source shape deviates from a regular sphere. The best constraints are obtained for GW signals at high frequencies.
Prospects
SN2023ixf is one of the most interesting CCSN events recorded in the last years to search for the GW emission. Unfortunately it happened when the network of ground based GW detectors were not all operational and we have GW data for only 15% of all possible times of the collapse. This limits the chance of detection.
Assuming the collapse happened when the two LIGO detectors were in observing mode, SN 2023ixf allows us to constrain the parameter space for GW emission models. However, the best prospects for a GW detection with the current generation of GW detectors favor a CCSN within the Local group.
Find out more:
Back to the overview of science summaries.