We report the most sensitive search to date for continuous gravitational waves from Scorpius X-1, a neutron star in a binary orbit with a low-mass star. Using data from Advanced LIGO’s first observing run (O1), along with an improved search method, we were able to achieve a sensitivity seven times better than the best initial LIGO search. We did not detect gravitational waves from Sco X-1, but we placed limits on their strength that are within a factor of between 1.2 and 3.5 of some predictions, depending on assumptions.
The binary black hole mergers GW150914 and GW151226, observed by LIGO in 2015, were examples of transient gravitational waves: relatively strong signals which were observable in our detectors for only a few seconds. We also look for continuous gravitational waves, fainter signals which are “always on.” We try to find these by adding up their effects over the entire observing run.
The most likely source of a continuous signal in LIGO is a neutron star, spinning tens to hundreds of times a second. If the neutron star has a small irregularity, it will give off gravitational radiation. Unlike the “chirp” of a binary merger, this will be more like a pure musical note at one frequency. As the Earth rotates on its axis and moves along its orbit, the frequency at which we receive the note will change due to the Doppler effect. When we do a targeted search for gravitational waves from pulsars (neutron stars known from their pulsed “lighthouse” emission), we know just what note to “listen” for. When we search for unknown neutron stars, we need to try all frequencies and Doppler shifts. The situation in between is a directed search for a known neutron star which we don’t see as a pulsar. The targeted and directed searches are examples of multi-messenger astronomy, where we use electromagnetic observations of a neutron star to inform the search for gravitational waves from that object.
One promising type of directed search is for a low-mass X-ray binary (LMXB), in which a neutron star pulls matter off of its lower-mass companion star while the two orbit their center of mass. The star-stuff falling (“accreting”) onto the neutron star can make it spin faster. In fact, this is how the fastest-spinning neutron stars are believed to have been “spun up.” Eventually, something else, such as emission of gravitational waves or interaction with the neutron star’s magnetic field, will balance out this accretion spinup, and the spin will stay in approximate equilibrium.
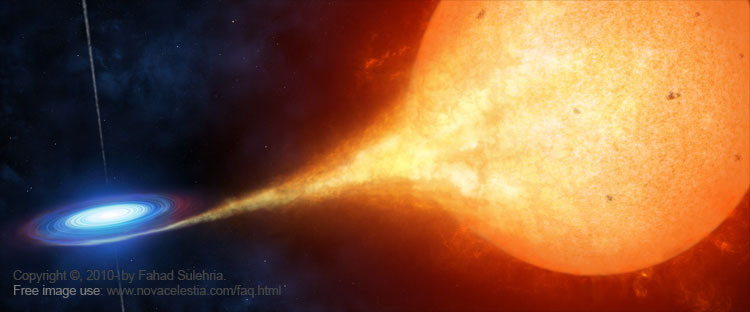
An artist’s impression of a low-mass X-ray binary like Scorpius X-1. (Astronomical Illustrations and Space Art, by Fahad Sulehria, http://www.novacelestia.com/)
Scorpius X-1 (Sco X-1) is the brightest LMXB; in fact, it’s the second brightest object seen when observing the sky in X-rays, after the Sun. Sco X-1 is relatively nearby within our galaxy, only 9,000 light years away. From the strength of the X-ray signal, we can estimate the potential strength of continuous gravitational waves. In the best-case scenario, where gravitational waves completely offset the accretion spinup, we can predict what’s known as the torque balance level. Sco X-1’s torque balance level is higher than that of any other LMXB, and it has been the subject of a number of directed searches by the LIGO Scientific Collaboration and Virgo Collaboration. These include the “radiometer” search which looks for correlations in the outputs of different detectors at the same time, with minimal assumptions about the signal, and the “Viterbi” method, based on the earlier “sideband” method, which takes advantage of the changes of the signal caused by the additional Doppler shift from the binary orbit.
The cross-correlation method uses the waveform model for a rotating neutron star to look for correlations in data taken not just at the same time in different detectors, but at different times in either the same or different detectors. To do this, we need to use properties of the signal, such as the frequency (number of cycles per second) of the gravitational waves, the direction to the neutron star (its “sky position”), and the parameters of the neutron star’s orbit (period, radius, time at which the neutron star passes a reference point), which determine the Doppler shift of the signal. Since we don’t know these, we have to try out different possible values and see if any produce significant correlations. The search only considers correlations up to some specified time offset. We can make the search more sensitive (but slower) by increasing this offset, or quicker (but less sensitive) by decreasing it. In practice, to maximize the chance of a detection (or an interesting upper limit), we spread computing costs unevenly over possible signal parameters. In this search with O1 data, the maximum offset varied from 240 seconds to 25,000 seconds.
We can also use this adjustable offset to follow up potential signals. If a set of parameters produces a cross-correlation larger than expected from just random detector noise, described by the signal-to-noise ratio (SNR), we can analyze the data with the same parameters, increasing the maximum allowed offset, and see if the observed SNR increases as expected from the signal model. In particular, we can perform the same analysis on detector data with simulated signals added and see how their SNRs increase. We found that none of the potential signals from the search of O1 data increased its SNR to the degree seen for simulated signals.
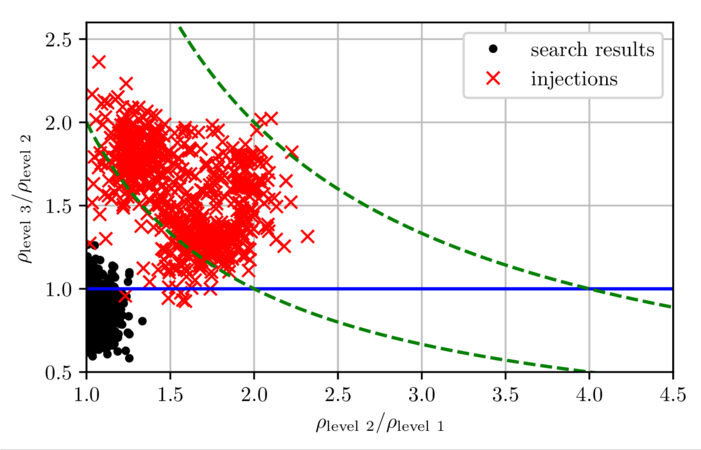
This figure shows the results of follow-up analyses of potential signals from the search. The x-axis shows the ratio of detection statistics before and after a quadrupling of the maximum time offset in the cross-correlation, which should theoretically double the SNR. The y-axis shows the ratio of SNRs before and after a second quadrupling. (The green dashed lines show constant values of x times y, i.e., the ratio of SNRs before and after the two quadruplings taken together.) The simulated signal injections (red x symbols) increase their SNRs much more than the candidates from the search (black dots).
Since we did not see any convincing candidate signals, we set upper limits on the strength of gravitational waves from Sco X-1, using the simulated signal results. The 95% upper limit at each frequency is the signal strength at which we’d expect 95% of the possible signals to produce a larger SNR than we observed at the same parameter values in the actual search. These upper limits are a factor of seven better than the best upper limits from initial LIGO (such as the S5 radiometer and “sideband” results), and a factor of three or four better than the other limits set in O1 by the radiometer and “Viterbi” searches.
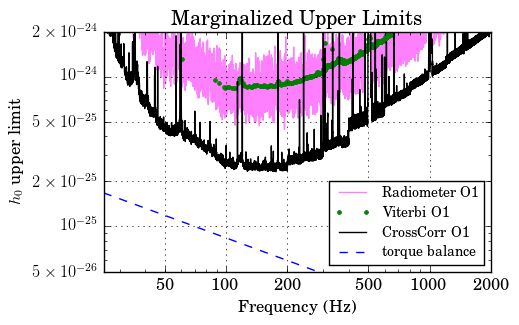
This figure shows the 95% upper limits set on h0 by the cross-correlation search, along with the results of the O1 radiometer and “Viterbi” searches. The cross-correlation results represent a factor of 3-4 improvement over these and a factor of about 7 over the best initial LIGO results. The torque balance line is an optimistic expected signal strength inferred from the X-ray flux from Sco X-1; our upper limits are still a factor of 3.4 or more above that.
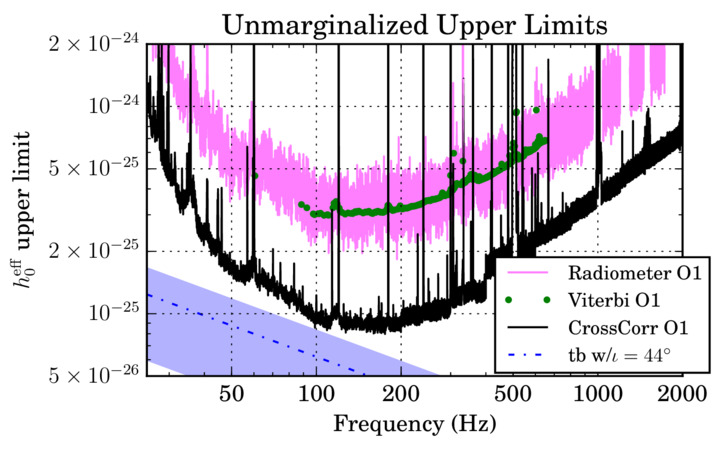
This figure shows the 95% upper limits set on h0eff by the cross-correlation search, along with the results of the O1 radiometer and “Viterbi” searches. This depends on both h0 and the unknown inclination angle iota. In the best-case of circularly polarized alignment, h0eff=h0. The torque balance line is shown for the range of possible inclinations. The upper limits are between 1.2 and 3.5 above the torque balance line.
Since the signal seen in our detectors depends on both the strain amplitude of the waves and the inclination angle defining the orientation of the axis about which the neutron star rotates, we have two ways of handling the unknown inclination:
- The unmarginalized upper limit can be thought of as an upper limit assuming the most favorable orientation, where we’re looking right down the neutron star’s axis. It can be converted to the assumption of any other inclination by multiplying it by a constant factor. Depending on the inclination assumption, the upper limits are between 1.2 and 3.5 of the torque balance level. If we assume the neutron star’s spin is aligned perpendicular to its orbit, the upper limits are 1.7 times the torque balance level at 100 Hertz.
- The marginalized upper limit lets the set of possible signals at a given strain amplitude include a full range of inclinations; since we’re looking for the level where we find 95% of them, this is dominated by the worst case scenario. If we compare this marginalized upper limit to the torque balance prediction, we see that the upper limits are a factor 3.4 higher than this level at a signal frequency of 100 Hertz.
LIGO and Virgo will take more sensitive data in their upcoming observing runs, and we are continuing to make improvements to our search methods. We expect that during the time of Advanced LIGO and Advanced Virgo, our sensitivity to gravitational waves from Sco X-1 will surpass the torque balance level, depending on the frequency of the signal. Detecting these waves would open up a new observation to the physics of neutron stars and accretion. More sensitive upper limits could begin to rule out possible equilibrium scenarios for Sco X-1, just like previous “multi-messenger” LIGO/Virgo observations such as the constraints on gravitational waves from the gamma-ray bursts GRB070201 and GRB051103, and the Crab and Vela pulsars.
Read more:
- Freely readable preprint of the paper describing the details of the full analysis and results: Upper Limits on Gravitational Waves from Scorpius X-1 from a Model-Based Cross-Correlation Search in Advanced LIGO Data
- Freely readable preprints of the papers describing the method in more detail: